Fault-tolerant quantum computing 'will solve exciting science problems'
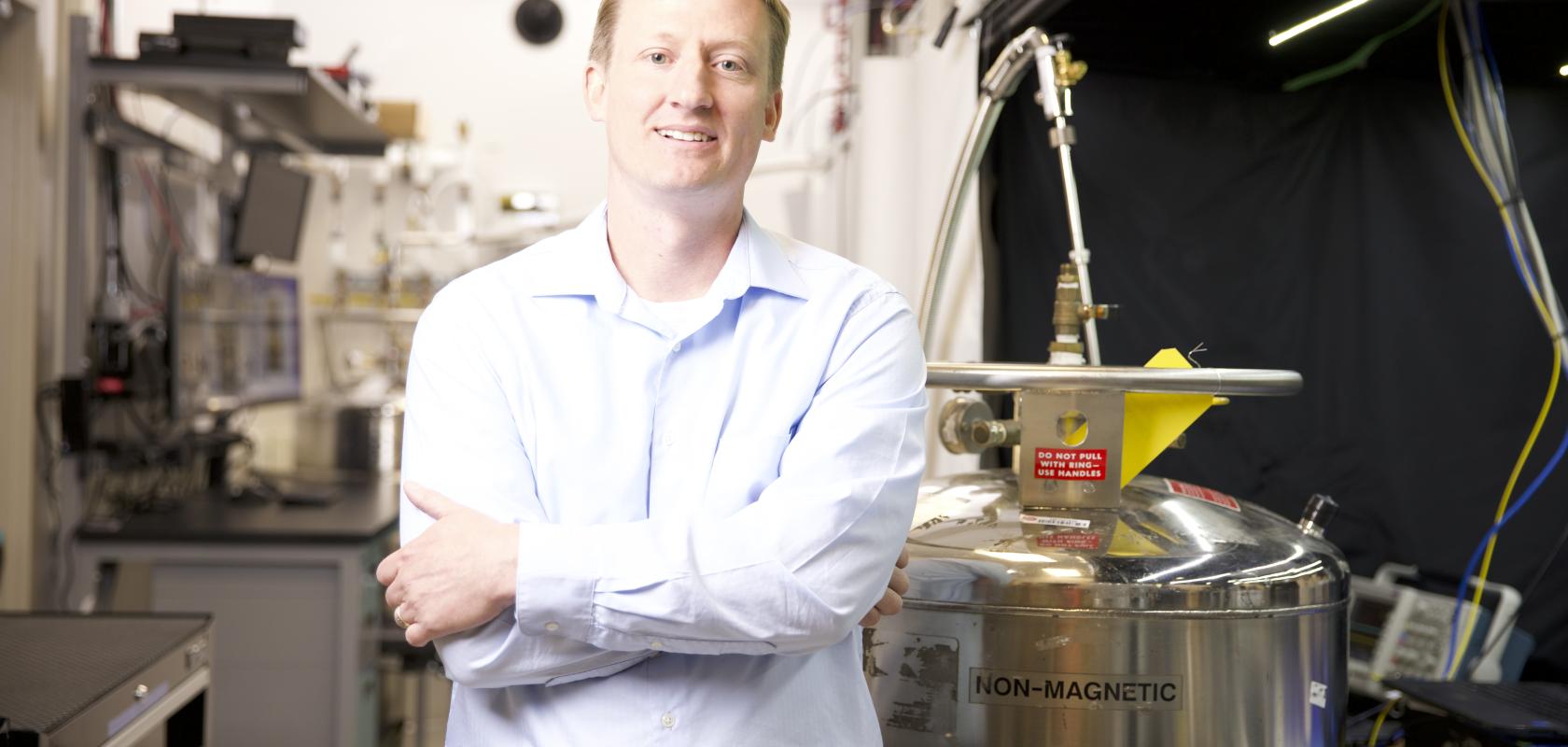
Credit: Quantinuum
Quantinuum’s Dr Harry Buhrman and Russell Stutz discuss the technological innovation underpinning the company’s development of fault-tolerant quantum computing systems
Register for FREE to keep reading
Join 12,000+ scientists, engineers, and IT professionals driving innovation through informatics, HPC, and simulation with:
- Insights into HPC, AI, lab informatics & data
- Curated content for life sciences, engineering & academia
- Access to Breakthroughs: real-world computing success
- Free reports & panels, including the Lab Informatics Guide
- White Papers & software updates for smarter research
Sign up now
Already a member? Log in here
Your data is protected under our privacy policy.
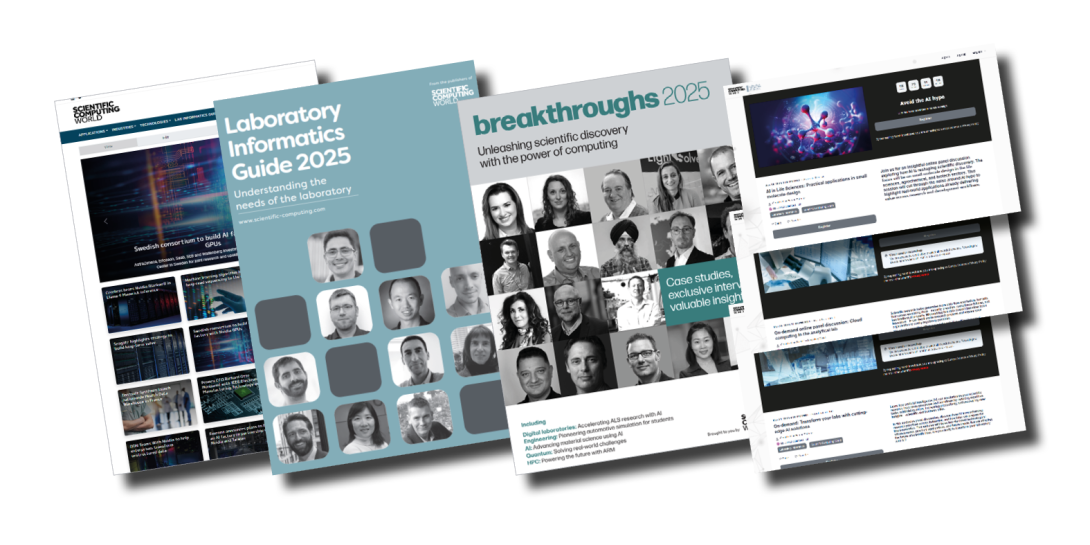